Dark Matter: Discerning What We Cannot See
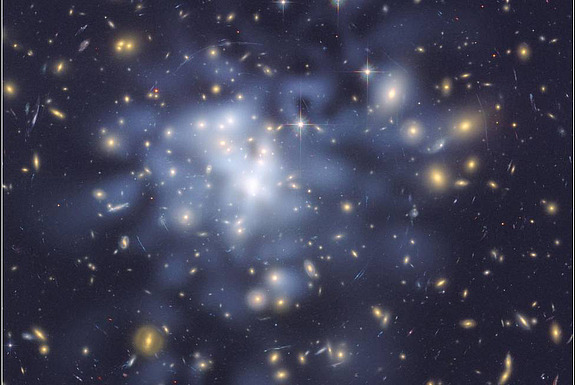
The field of cosmology has come a long way toward understanding the universe as a physical system: we’ve mapped its history and structure in great detail, we know what it went through since a few seconds after its birth until today, we understand what sources its light, and we have a grip on the math describing the laws of gravity that govern the cosmic structures we observe through telescopes. Yet, most of its content is locked up within the “dark sector” we cannot see and do not yet understand.
When I was a graduate student at Caltech, Annika Peter, a senior postdoc at the time (now faculty at the Ohio State University), made a descriptive comparison between the universe and a cupcake: “The dough that makes up most of it, that’s the dark energy [the stuff that causes it to expand at an accelerating pace]. The icing is dark matter. And the sprinkles on top––that’s all the ordinary matter that we know and love.” At the Institute, I study the dark matter “icing” that keeps together all the stars and galaxies with its gravity, but does little else––it does not seem to emit or absorb light, nor do its constituents seem to collide much with ordinary particles. Specifically, I design and apply new ways to probe this mysterious substance, using a combination of theoretical and analysis tools, and data from a variety of observations and measurements. Here, I will first give you a brief summary of the status of the field of dark matter research and then explain some of my most recent work.
We know that dark matter makes up about 80 percent of the total matter content of the universe because we observe how its gravitational pull affects normal matter (“baryons”) in a wide range of astrophysical systems. In the local universe, we see nearby galaxies (and our own) spinning at a far faster rate than if only stars and gas held them together, implying the presence of an additional unseen source of gravity. More distantly, we observe that clusters of galaxies bend the trajectories of light coming from even more distant objects (the effect known as gravitational lensing), far more prominently than their luminous matter alone could. And from the very dawn of the cosmos, the cosmic microwave background (CMB) radiation––a relic light emitted when the universe was only 400,000 years old, and coming at us from all over the sky––would have far more directional irregularity, or anisotropy, if this dark matter were absent or made up of ordinary baryons. Most importantly, all these (and many more) different pieces of observational evidence give a very precise quantitative picture of how much dark matter there is, and how it is distributed––and all of them agree. It turns out that dark matter has been around since before the CMB was emitted, and today every galaxy lives within a heavy dark matter blob we call its “halo.”
Leading theories of dark matter involve a new kind of fundamental particle, or particles (though there are more exotic proposals, for example, involving dark matter made out of “primordial black holes”… but I will only discuss particle physics models here). There are many such theories, but most of them predict some amount of interaction between dark matter and the known particles. Mapping out the basic properties of dark matter constituents––their mass, charge, spin––and understanding how they interact (between each other and with baryons) is one of the main open quests in modern physics. Many avenues of probing dark matter have been explored to date. Some of them attempt to detect photons or electrons that would be produced if dark matter particles collide and annihilate in centers of galaxies, such as our own. Others search for traces of dark matter particle production in collider experiments (like the Large Hadron Collider in Geneva). Traditionally, however, the most sensitive searches for dark matter particles have been the so-called direct detection experiments.
Direct detection experiments look for rare collisions of dark matter with nuclei in experimental targets (large chambers with xenon, germanium crystals, etc.) in underground detectors. They rely on the idea that, as the Sun rotates around the galactic center, it sweeps its way through the sea of dark matter that is our own dark matter halo, subjecting the Earth––and the detectors in its underground labs––to a “dark matter wind.” As dark matter appears very small to baryons (in technical terms, the cross section for collisions between dark matter and nuclei must be very, very small, otherwise we would have already detected it), the extensive shielding of the experiments does not stop it, and the elusive particles pass straight through the earth and the detectors. Nearly always, this goes unnoticed, even though there are about a hundred million of them passing through each of us every second, if dark matter is ten times heavier than a proton (and even more if it is lighter)! Very rarely though one might bump into a target nucleus and be detected that way. Current-generation experiments (several dozen of them scattered worldwide, named LUX, Xenon, CDMS, PICO, etc.) have yet to make a detection. A new generation of bigger targets and more sensitive measurements is forthcoming, and direct detection of dark matter might be around the corner.
In light of this, part of my work at the Institute has focused on understanding, by means of numerical computer simulations and analysis, what information we may learn from these experiments. One important conclusion I came to was that, even if the signal they see turns out to be as strong as possible (given that none of the current experiments have yet seen it), we might be able to measure the mass of a dark matter particle, but we will not be able to use this data to confidently confirm or falsify specific dark matter theories. The data will not have enough statistical power to address such interesting questions. We thus must search for alternative and complementary ways to probe the physical nature of dark matter.
That brings me to another important point about these traditional searches for dark matter particles: the nuclear measurements they are making cannot detect collisions with particles lighter than the proton. The absence of a signal to date might then simply be due to dark matter’s mass lying below the current detection thresholds. As a cosmologist, I use cosmological and astrophysical observations, and in particular the observations of the CMB, in order to test fundamental physics. It was natural for me to turn to thinking about ways that the CMB measurements can be used to search for evidence of dark matter particle physics. Before I explain how, let me first give you a brief overview of what the CMB actually is, and what it can tell us.
Shortly after the Big Bang, the universe was a fairly uniform, dense, and hot mix of particles, all moving together. In this mix were photons, protons, electrons, and dark matter (and traces of other things). Protons and electrons were in a state of acoustic oscillation: gravity from dark matter clumps would pull them, and they would compress, heat up, and then expand under the pressure of photons that were pushing on the electrons in the plasma; this expansion would in turn cool them down again, and the process would repeat. If you imagine oscillations of a ball hanging on a spring, the gravitational field of the Earth would be analogous to that of dark matter clumps in the early universe, and the restoring force of the photons is like the elasticity of the spring. The two acting together make a ball oscillate up and down––or, in our case, the baryons compress and rarify in a cyclic way.
Once the universe expanded and cooled enough (to a temperature close to that of a star’s surface), the photons were set free to travel all the way to the present day, and into our observatories––these are the CMB photons. And the image they depict in the sky––the tiny differences in their temperatures in different directions––reflect the processes that were happening in this early universe.
Now, if dark matter scatters off protons today, it was doing so even more often at early times, when the universe was tightly packed with fast-moving particles. As a result of these collisions, dark matter clumps collapsing under gravity would exert a friction force on the oscillating baryons––imagine dunking our ball on a spring into a bucket of water. The friction damps the oscillations, especially the high-frequency waves. The damping effect would be captured by the CMB light as an unusual lack of small irregularities––“smoothing”––in its temperature in the sky. In technical terms, the power spectrum of the CMB temperature (and polarization) would show a suppression on small angular scales.
While studying this effect, in collaboration with dark matter physicist Kimberly Boddy from Johns Hopkins University, I have very recently performed the first full cosmological search for evidence of dark matter particle collisions in the early universe, using data from the Planck satellite. In a series of studies, we have been able to probe particles as light as one thousandth of electron mass (a million times lighter than the typical sensitivity threshold of direct detection experiments). We also used the formulation of the so-called “effective theory of dark matter interactions” to systematically categorize all possible interaction theories and constrain them. We are now preparing to apply this analysis to state-of-the art CMB measurements made by the Atacama Cosmology Telescope collaboration (based at Princeton), in order to further push the bounds on dark matter interaction physics.
So far, we haven’t found the signal we are looking for. But a tremendous amount of data is imminently coming from galaxy surveys and from the next-generation CMB measurements that will make cosmological observations one of the main avenues for testing fundamental physics. With my recent work at the Institute, we were able to peer back at what fundamental particles were doing when the universe was only two years old. . . we can now dare to imagine what happens even before.